1.3: Biodiesel from Oils and Fats
- Page ID
- 46840
\( \newcommand{\vecs}[1]{\overset { \scriptstyle \rightharpoonup} {\mathbf{#1}} } \)
\( \newcommand{\vecd}[1]{\overset{-\!-\!\rightharpoonup}{\vphantom{a}\smash {#1}}} \)
\( \newcommand{\id}{\mathrm{id}}\) \( \newcommand{\Span}{\mathrm{span}}\)
( \newcommand{\kernel}{\mathrm{null}\,}\) \( \newcommand{\range}{\mathrm{range}\,}\)
\( \newcommand{\RealPart}{\mathrm{Re}}\) \( \newcommand{\ImaginaryPart}{\mathrm{Im}}\)
\( \newcommand{\Argument}{\mathrm{Arg}}\) \( \newcommand{\norm}[1]{\| #1 \|}\)
\( \newcommand{\inner}[2]{\langle #1, #2 \rangle}\)
\( \newcommand{\Span}{\mathrm{span}}\)
\( \newcommand{\id}{\mathrm{id}}\)
\( \newcommand{\Span}{\mathrm{span}}\)
\( \newcommand{\kernel}{\mathrm{null}\,}\)
\( \newcommand{\range}{\mathrm{range}\,}\)
\( \newcommand{\RealPart}{\mathrm{Re}}\)
\( \newcommand{\ImaginaryPart}{\mathrm{Im}}\)
\( \newcommand{\Argument}{\mathrm{Arg}}\)
\( \newcommand{\norm}[1]{\| #1 \|}\)
\( \newcommand{\inner}[2]{\langle #1, #2 \rangle}\)
\( \newcommand{\Span}{\mathrm{span}}\) \( \newcommand{\AA}{\unicode[.8,0]{x212B}}\)
\( \newcommand{\vectorA}[1]{\vec{#1}} % arrow\)
\( \newcommand{\vectorAt}[1]{\vec{\text{#1}}} % arrow\)
\( \newcommand{\vectorB}[1]{\overset { \scriptstyle \rightharpoonup} {\mathbf{#1}} } \)
\( \newcommand{\vectorC}[1]{\textbf{#1}} \)
\( \newcommand{\vectorD}[1]{\overrightarrow{#1}} \)
\( \newcommand{\vectorDt}[1]{\overrightarrow{\text{#1}}} \)
\( \newcommand{\vectE}[1]{\overset{-\!-\!\rightharpoonup}{\vphantom{a}\smash{\mathbf {#1}}}} \)
\( \newcommand{\vecs}[1]{\overset { \scriptstyle \rightharpoonup} {\mathbf{#1}} } \)
\( \newcommand{\vecd}[1]{\overset{-\!-\!\rightharpoonup}{\vphantom{a}\smash {#1}}} \)
\(\newcommand{\avec}{\mathbf a}\) \(\newcommand{\bvec}{\mathbf b}\) \(\newcommand{\cvec}{\mathbf c}\) \(\newcommand{\dvec}{\mathbf d}\) \(\newcommand{\dtil}{\widetilde{\mathbf d}}\) \(\newcommand{\evec}{\mathbf e}\) \(\newcommand{\fvec}{\mathbf f}\) \(\newcommand{\nvec}{\mathbf n}\) \(\newcommand{\pvec}{\mathbf p}\) \(\newcommand{\qvec}{\mathbf q}\) \(\newcommand{\svec}{\mathbf s}\) \(\newcommand{\tvec}{\mathbf t}\) \(\newcommand{\uvec}{\mathbf u}\) \(\newcommand{\vvec}{\mathbf v}\) \(\newcommand{\wvec}{\mathbf w}\) \(\newcommand{\xvec}{\mathbf x}\) \(\newcommand{\yvec}{\mathbf y}\) \(\newcommand{\zvec}{\mathbf z}\) \(\newcommand{\rvec}{\mathbf r}\) \(\newcommand{\mvec}{\mathbf m}\) \(\newcommand{\zerovec}{\mathbf 0}\) \(\newcommand{\onevec}{\mathbf 1}\) \(\newcommand{\real}{\mathbb R}\) \(\newcommand{\twovec}[2]{\left[\begin{array}{r}#1 \\ #2 \end{array}\right]}\) \(\newcommand{\ctwovec}[2]{\left[\begin{array}{c}#1 \\ #2 \end{array}\right]}\) \(\newcommand{\threevec}[3]{\left[\begin{array}{r}#1 \\ #2 \\ #3 \end{array}\right]}\) \(\newcommand{\cthreevec}[3]{\left[\begin{array}{c}#1 \\ #2 \\ #3 \end{array}\right]}\) \(\newcommand{\fourvec}[4]{\left[\begin{array}{r}#1 \\ #2 \\ #3 \\ #4 \end{array}\right]}\) \(\newcommand{\cfourvec}[4]{\left[\begin{array}{c}#1 \\ #2 \\ #3 \\ #4 \end{array}\right]}\) \(\newcommand{\fivevec}[5]{\left[\begin{array}{r}#1 \\ #2 \\ #3 \\ #4 \\ #5 \\ \end{array}\right]}\) \(\newcommand{\cfivevec}[5]{\left[\begin{array}{c}#1 \\ #2 \\ #3 \\ #4 \\ #5 \\ \end{array}\right]}\) \(\newcommand{\mattwo}[4]{\left[\begin{array}{rr}#1 \amp #2 \\ #3 \amp #4 \\ \end{array}\right]}\) \(\newcommand{\laspan}[1]{\text{Span}\{#1\}}\) \(\newcommand{\bcal}{\cal B}\) \(\newcommand{\ccal}{\cal C}\) \(\newcommand{\scal}{\cal S}\) \(\newcommand{\wcal}{\cal W}\) \(\newcommand{\ecal}{\cal E}\) \(\newcommand{\coords}[2]{\left\{#1\right\}_{#2}}\) \(\newcommand{\gray}[1]{\color{gray}{#1}}\) \(\newcommand{\lgray}[1]{\color{lightgray}{#1}}\) \(\newcommand{\rank}{\operatorname{rank}}\) \(\newcommand{\row}{\text{Row}}\) \(\newcommand{\col}{\text{Col}}\) \(\renewcommand{\row}{\text{Row}}\) \(\newcommand{\nul}{\text{Nul}}\) \(\newcommand{\var}{\text{Var}}\) \(\newcommand{\corr}{\text{corr}}\) \(\newcommand{\len}[1]{\left|#1\right|}\) \(\newcommand{\bbar}{\overline{\bvec}}\) \(\newcommand{\bhat}{\widehat{\bvec}}\) \(\newcommand{\bperp}{\bvec^\perp}\) \(\newcommand{\xhat}{\widehat{\xvec}}\) \(\newcommand{\vhat}{\widehat{\vvec}}\) \(\newcommand{\uhat}{\widehat{\uvec}}\) \(\newcommand{\what}{\widehat{\wvec}}\) \(\newcommand{\Sighat}{\widehat{\Sigma}}\) \(\newcommand{\lt}{<}\) \(\newcommand{\gt}{>}\) \(\newcommand{\amp}{&}\) \(\definecolor{fillinmathshade}{gray}{0.9}\)B. Brian He
Biological Engineering
University of Idaho
Moscow, ID, USA
Scott W. Pryor
Agricultural and Biosystems Engineering and College of Engineering
North Dakota State University, Fargo, ND, USA
Key Terms |
Feedstocks | Conversion process | Properties |
Chemistry | Process configuration | Storage and handling |
Introduction
Biodiesel is the term given to a diesel-like fuel made from biologically derived lipid feedstocks, such as vegetable oils, animal fats, and their used derivatives such as waste cooking oils. Biodiesel is a renewable fuel that can be made from a diverse array of domestic feedstocks, has low safety concerns for use and handling, and can have relatively low environmental impact from production and use.
Biodiesel has several properties that make it a safer fuel than conventional petroleum-based diesel. While conventional diesel is categorized as a flammable fuel, biodiesel is rated as combustible, which means it has a low vapor pressure, is resistant to static sparks, and is much less likely to self-ignite during storage. During transportation, tankers carrying pure biodiesel are not required to display warning signs in the United States.
Biodiesel is especially of interest to farmers because of the potential for on-farm production using harvested crops. Oil can be extracted from oilseeds relatively easily, and this oil can then be used to make biodiesel to run farm machinery. It provides farmers an additional resource for economic welfare and an additional choice for managing cropland. In addition, using biodiesel from domestically grown feedstocks can decrease a country’s dependence on imported oil, thus enhancing national energy security. On the other hand, concerns are sometimes raised about converting oils and fats, which could serve as food resources, into fuels (Prasad and Ingle, 2019).
Biodiesel is typically considered an environmentally friendly fuel. Production and combustion of biodiesel results in less air pollution than using conventional diesel. According to a study sponsored by the U.S. Department of Agriculture and the Department of Energy, using biodiesel in urban buses can reduce total particulate matter (PM), carbon monoxide (CO) and sulfur oxides (SOx) by 32%, 35% and 8%, respectively (Sheehan et al., 1998).
The diesel engine is named for Rudolf Diesel, who invented it in the 1890s. Diesel’s engines could run on various fuels including vegetable oils. At the Paris Exposition in 1900, Diesel demonstrated his engines running on peanut oil and made this famous statement:
The use of vegetable oils for engine fuels may seem insignificant today. But such oils may become in course of time as important as petroleum and the coal tar products of the present time.
Diesel’s vision was valid in that vegetable oils can still be used directly as a fuel for diesel engines. However, raw vegetable oils without pre-processing are not an ideal fuel for modern diesel engines due to their high viscosity and other chemical properties. Burning raw vegetable oils in today’s diesel engines results in heavy carbon deposits in the cylinders, which can stall the engine in a short period of time.
To overcome this problem, research was conducted starting in the late 1930s to chemically process vegetable oils into a mixture of short-chained alkyl fatty acid esters. This fuel has a much lower viscosity and is thus better suited for use in diesel engines. During the petroleum crisis in the 1970s, the use of alkyl fatty acid esters as a fuel for diesel engines became more popular. Two decades later, in the 1990s, the name “biodiesel” was coined and gained popularity.
In the early 1980s, Mittelbach and his team at the Technical University of Graz in Austria were the first to research biodiesel as a diesel fuel. The commercialization of biodiesel started with a pilot biodiesel production facility by an Austrian company, Gaskoks, in 1987. The European Biodiesel Board (EBB), a non-profit organization promoting the use of biodiesel in Europe, was founded in 1997.
Biodiesel research and utilization in the U.S. started around the same time as in Europe. Dr. Charles Peterson and his research team at the University of Idaho conducted a series of research projects on the use of vegetable oil as tractor fuel. The team worked on biodiesel production, engine testing, emission assessment, and field utilization. The National Biodiesel Board (NBB) was founded in the U.S. in 1992 and has conducted health and environmental assessments on biodiesel utilization. The NBB also registered biodiesel with the U.S. Environmental Protection Agency (USEPA) as a substitute fuel for diesel engines. Supported by the NBB and the biodiesel research community, biodiesel was established as an industry sector. Total biodiesel production reached approximately 7.2 billion L in the USA in 2018 with an additional 39.4 billion L produced globally.
Although biodiesel can be used as a pure diesel-replacement fuel called B100, it is typically available as a diesel/ biodiesel blend at retail pumps. Biodiesel blends are designated to indicate a volumetric mixture such as B5 or B20 for 5% or 20% biodiesel, respectively, in conventional diesel.
Outcomes
After reading this chapter, you should be able to:
- • Describe the advantages and limitations of using biodiesel in diesel-powered engines
- • Describe biodiesel production processes
- • Explain how biodiesel is similar to and different from conventional petroleum-based diesel
- • Describe how feedstock composition and properties affect biodiesel properties
- • Explain the important unit operations commonly used for producing biodiesel
- • Calculate proportions of vegetable oil, methanol, and catalyst needed to make a given quantity of biodiesel, and the size of the reactor required for conversion
Concepts
Biodiesel Chemistry
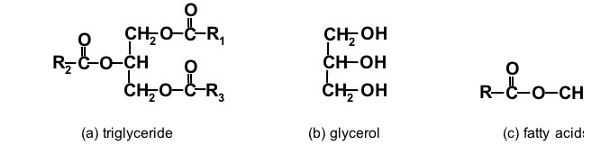
To qualify as biodiesel in the U.S., a fuel must strictly comply with the ASTM definition of a “fuel comprised of mono-alkyl esters of long chain fatty acids derived from vegetable oils or animal fats, designated B100” (ASTM, 2015). It must also meet all of the quality parameters identified in that standard. In Europe, the definition of biodiesel is covered by the European standard EN 14214 (CEN, 2013). The generic name for vegetable oils (more generally plant oils) or animal fats issimply fat or lipid. The primary distinguishing factor between a fat and an oil is that a fat is a solid at room temperature while an oil is a liquid. The primary compounds in both oils and fats are a group of chemicals called triglycerides (Figure \(\PageIndex{1a}\)).
Glycerol (Figure \(\PageIndex{1b}\)), also known as glycerin, is a poly-hydric alcohol with three alcoholic hydroxyl groups (-OH). Pure glycerol is colorless, odorless, and hygroscopic. Fatty acids (Figure \(\PageIndex{1c}\)) are a family of carboxylic acids with relatively long carbon chains.
Triglycerides, also called triacylglycerols, are the glycerol esters of fatty acids, in which three fatty acids attach chemically to a glycerol carbon backbone where the hydroxyl (OH) groups are attached. Triglycerides in oils and fats may contain fatty acid chains of 10 to 24 carbons (C10-C24) but are most commonly 16 to 18 carbons (C16-C18) in length. The three fatty acids attached to the glycerol molecule can be the same or different. The alkyl chain length of fatty acids, the presence and number of double bonds contained in the fatty acid chains, and the position and orientation of the double bonds collectively determine the chemical and physical properties of the triglyceride. Some examples are provided in Table \(\PageIndex{1}\).
Abbreviation | Common Name | Formula | Chemical Structure | MW[1] |
---|---|---|---|---|
C12:0[2] |
lauric acid |
C12H24O2 |
CH3(CH2)10COOH |
200.3 |
C14:0 |
myristic acid |
C14H28O2 |
CH3(CH2)12COOH |
228.4 |
C16:0 |
palmitic acid |
C16H32O2 |
CH3(CH2)14COOH |
256.5 |
C18:0 |
stearic acid |
C18H36O2 |
CH3(CH2)16COOH |
284.5 |
C18:1 |
oleic acid |
C18H34O2 |
CH3(CH2)7CH:CH(CH2)7COOH |
282.5 |
C18:2 |
linoleic acid |
C18H32O2 |
CH3(CH2)3(CH2CH:CH)2(CH2)7COOH |
280.5 |
C18:3 |
linolenic acid |
C18H30O2 |
CH3(CH2CH:CH)3(CH2)7COOH |
278.5 |
C20:0 |
arachidic acid |
C20H40O2 |
CH3(CH2)18COOH |
312.6 |
C20:1 |
eicosenoic acid |
C20H38O2 |
CH3(CH2)7CH:CH (CH2)9COOH |
310.5 |
C20:5 |
eicosapentaenoic |
C20H30O2 |
CH3(CH2CH:CH)5(CH2)3COOH |
302.5 |
C22:1 |
erucic acid |
C22H42O2 |
CH3(CH2)7CH:CH(CH2)12COOH |
338.6 |
[1] MW = molecular weight, g/mol
[2] Cx:y stands for a chain of x carbon atoms with y double bonds in that chain.
Biodiesel Properties
Biodiesel is a commercialized biofuel used by consumers around the globe. Several international standards have been developed and approved to assure engine manufacturers and diesel engine customers that biodiesel meets specified fuel quality requirements. As a commercial product, biodiesel must comply with the specifications defined by the ASTM Standard D6751 (ASTM, 2015) in North America or EN14214 (CEN, 2013) in Europe. Several other countries have also developed their own standards; in many cases, they are based on the ASTM and EN standards. Table \(\PageIndex{2}\) summarizes the specifications for biodiesel fuel according to these two standards.
Biodiesel properties are affected by both the feedstock and the conversion process. Meeting specification for all parameters in the relevant standards must be documented before a fuel can be marketed. However, some fuel properties are more critical than others in terms of use. In the USA, biodiesel sulfur content must be no more than 15 ppm for Grade S15, and 500 ppm for Grade S500, to qualify as an ultra-low sulfur fuel. If virgin vegetable oils are used as the feedstock, sulfur content in the biodiesel is typically very low. However, if used cooking oils or animal fats are used, the sulfur content in biodiesel must be carefully monitored to meet the required specification.
Property | Units | ASTM D6751[a] | EN14214 | |
---|---|---|---|---|
Grade 1B (S15) |
Grade 2B (S15) |
|||
Sulfur (15 ppm or lower level) (maximum) |
ppm |
15 |
15 |
[b] |
Cold soak filterability (maximum) |
Sec. |
200 |
360 |
[b] |
Mono-glyceride (maximum) |
% mass |
0.40 |
[b] |
0.8 |
Calcium & magnesium combined (maximum) |
ppm (μg/g) |
5 |
5 |
|
Flash point (closed cup) (minimum) |
°C |
93 |
101 |
|
Alcohol control (one of the following shall be met) |
||||
a) Methanol content (maximum) |
mass % |
0.2 |
0.2 |
|
b) Flash point (minimum temperature) |
°C |
130 |
[b] |
|
Water and sediment (maximum) |
% volume |
0.050 |
0.005 |
|
Kinematic viscosity (40°C) |
mm2/s |
1.9–6.0 |
3.5–5.0 |
|
Sulfated ash (maximum) |
% mass |
0.02 |
0.02 |
|
Copper strip corrosion |
No. 3 |
No. 1 |
||
Cetane number (minimum) |
47 |
51 |
||
Cloud point |
°C |
Must be reported |
[b] |
|
Carbon residue (maximum) |
% mass |
0.05 |
0.03 |
|
Acid number (maximum) |
mg KOH/g |
0.50 |
0.5 |
|
Free glycerol (maximum) |
% mass |
0.02 |
0.02 |
|
Total glycerol (maximum) |
% mass |
0.24 |
0.25 |
|
Phosphorus content (maximum) |
% mass |
0.001 |
0.001 |
|
Distillation temperature (90%) (maximum) |
°C |
360 |
[b] |
|
Sodium and potassium combined (maximum) |
ppm (μg/g) |
5 |
5 |
|
Oxidation stability (minimum) |
hours |
3 |
6 |
[a] Grade refers to specification for monoglycerides and cold soak filterability. S15 indicates maximum sulphur content of 15 ppm.
[b] Not specified in the standard
A liquid fuel’s flash point refers to the lowest temperature at which its vapor will be combustible. Biodiesel has a high flash point, making it safe for handling and storage. The flash point, however, may drop if the residual alcohol from the biodiesel production process is inadequately removed. To maintain a high flash point, biodiesel alcohol content cannot be more than 0.2%. Cloud point and cold soak filterability are both properties relating to flowability at cold temperatures and are important for biodiesel use in relatively low temperature environments. Cloud point refers to the temperature at which dissolved solids begin to precipitate and reduce clarity. Cold soak filterability refers to how well biodiesel flows through a filter at a specified temperature (4.4°C). Biodiesel is limited in its use in colder climates because it typically has a much higher cloud point (−6°C to 0°C for rapeseed and soybean based biodiesel and up to 14°C for palm oil based biodiesel) than conventional No. 2 diesel (−28°C to −7°C). Generally, methyl esters of long-chain, saturated fatty acids have high cloud points, especially in comparison to conventional diesel fuel. Although there are commercial additives available for improving biodiesel cold flow properties, their effectiveness is limited. Cold flow properties can be a limiting factor related to the biodiesel blend used (e.g., B2 vs. B10 or B20) in colder climates or at colder times of the year.
The presence of monoglycerides in biodiesel is an indicator of incomplete feedstock conversion and can adversely affect fuel combustion in an engine. Monoglycerides also contribute to measurements of both total glycerine and free glycerol. Total glycerol should be 0.24% or lower to avoid injector deposits and fuel filter clogging problems in engine systems.
Biodiesel viscosity is significantly lower than that of vegetable oil but is higher than conventional diesel in most cases. Biodiesel viscosity will vary based primarily on the fatty acid carbon chain length and level of saturation in the feedstock. Although specified biodiesel viscosity levels range from 2.8 to 6.1 mm2/s at 40°C, typical values are greater than 4 mm2/s at that temperature (Canackci and Sanli, 2008), while No. 2 conventional diesel has a specified viscosity range of 1.9–4.1 mm2/s at 40°C with typical values less than 3.0 mm2/s (ASTM, 2019).
Most biodiesel fuels have a higher cetane number than conventional diesel. Cetane number measures the ability of a fuel to ignite under pressure and a high cetane number is generally advantageous for combustion in diesel engines. Typical values are approximately 45–55 for soybean-based biodiesel and 49–62 for rapeseed-based biodiesel. The higher cetane number of biodiesel is largely attributed to the long carbon chain and high degree of unsaturation in fatty acid esters. Acid number of biodiesel fuel is an indication of free fatty acid content in biodiesel, which affects the oxidative and thermal stabilities of the fuel. To ensure biodiesel meets the specification of acid number, feedstocks with high free fatty acid content must be thoroughly treated and the finished product adequately washed.
Mineral ash contents of combined calcium and magnesium, combined sodium and potassium, and carbon residue have a harmful effect on biodiesel quality by leading to abrasive engine deposits. Phosphorus content is also regulated closely because of its adverse impact on the catalytic converter. Good quality control practices are vital in controlling residual mineral content in biodiesel. Biodiesel instability can also be affected negatively by excess water and sediment because of inadequate refining, or from contamination during transport or storage. Biodiesel tends to absorb moisture from the air, making it susceptible to such contamination. It can absorb 15–25 times more moisture than conventional petroleum-based diesel (He et al., 2007). Excess water can be controlled by adequately drying the moisture from biodiesel after water washing, and through proper handling and storage of the fuel.
Biodiesel Feedstocks
The primary feedstocks for making biodiesel are vegetable oils and animal fats. Typical properties are given in Table \(\PageIndex{3}\). The feedstocks for biodiesel production can be any form of triglycerides. The most commonly used feedstocks include soybean oil, rapeseed/canola oil, and animal fats. Used cooking oils and/or yellow/trap greases can also be used but may be better as supplements to a feedstock supply with more consistent quality and quantity. Feedstock choice for biodiesel production is generally based on local availability and price. Vegetable oils and/or animal fats all have existing uses and markets. The availability of each type of feedstock varies widely depending on current market conditions, and changes almost on a yearly basis. Before a biodiesel production facility is constructed, securing adequate feedstock supply is always the number one priority. Based on their availability, soybean oil and corn oil are the major feedstocks in the U.S., while rapeseed/canola oil is the most common feedstock used in Europe. Other major producing countries include Brazil and Indonesia which rely on soybean oil and palm oil, respectively.
Oils and Fats | Fatty Acid Profiles (% m/m) | |||||||
---|---|---|---|---|---|---|---|---|
C12:0 | C14:0 | C16:0 | C18:0 | C18:1 | C18:2 | C18:3 | C20:1 | |
Plant Oils | ||||||||
Algae oil |
12–15 |
10–20 |
4–19 |
1–2 |
5–8 |
35–48[2] |
||
Camelina |
12–15 |
15–20 |
30–40 |
12–15 |
||||
Canola, general |
1–3 |
2–3 |
50–60 |
15–25 |
8–12 |
|||
Canola, high oleic |
1–3 |
2–3 |
70–80 |
12–15 |
1–3 |
|||
Coconut oil |
45–53 |
16–21 |
7–10 |
2–4 |
5–10 |
1–2.5 |
||
Corn |
1–2 |
8–16 |
1–3 |
20–45 |
34–65 |
1–2 |
||
Cottonseed |
0–2 |
20–25 |
1–2 |
23–35 |
40–50 |
|||
Grape seed oil |
5–11 |
3–6 |
12–28 |
58–78 |
||||
Jatropha |
11–16 |
6–15 |
34–45 |
30–50 |
3–5[4] |
|||
Flax (linseed) oil |
4–7 |
2–4 |
25–40 |
35–40 |
25–60 |
|||
Mustard seed oil |
1–2 |
8–23 |
10–24 |
6–18 |
5–13 & 20–50[3] |
|||
Olive |
9–10 |
2–3 |
72–85 |
10–12 |
0–1 |
|||
Palm oil |
0.5–2 |
39–48 |
3–6 |
36–44 |
9–12 |
|||
Palm kernel oil |
45–55 |
14–18 |
6–10 |
1–3 |
12–19 |
|||
Peanut |
8–9 |
2–3 |
50–65 |
20–30 |
||||
Rapeseed (high erucic/oriental) |
1–3 |
0–1 |
10–15 |
12–15 |
8–12 |
45–60[3] & 7–10[4] |
||
Rapeseed (high oleic /canola) |
1–5 |
1–2 |
60–80 |
16–23 |
10–15 |
|||
Safflower (high linoleic) |
3–6 |
1–3 |
7–10 |
80–85 |
||||
Safflower (high oleic) |
1–5 |
1–2 |
70–75 |
12–18 |
0–1 |
|||
Sesame oil |
8–12 |
4–7 |
35–45 |
37–48 |
||||
Soybean oil |
6–10 |
2–5 |
20–30 |
50–60 |
5–11 |
|||
Soybean (high oleic) |
2–3 |
2–3 |
80–85 |
3–4 |
3–5 |
|||
Sunflower |
5–8 |
2–6 |
15–40 |
30–70 |
||||
Sunflower (high oleic) |
0–3 |
1–3 |
80–85 |
8–10 |
0–1 |
|||
Tung oil |
3–4 |
0–1 |
4–15 |
75–90 |
||||
Animal Fats | ||||||||
Butter |
7–10 |
24–26 |
10–13 |
28–31 |
1–3 |
0–1 |
||
Chicken fat |
||||||||
Lard |
1–2 |
25–30 |
10–20 |
40–50 |
6–12 |
0–1 |
||
Tallow |
3–6 |
22–32 |
10–25 |
35–45 |
1–3 |
[1] Compiled from various sources: Peterson et al., 1983; Peterson, 1986; Goodrum and Geller 2005; Dubois et al., 2007; Kostik et al., 2013; Knothe et al., 2015.
[2] C20:5
[3] C22:1
[4] C20:0
Compared to other oilseeds, soybeans have a relatively low oil content, typically 10–20% of the seed mass. However, soybean yields are relatively high, typically 2,500–4,000 kg/ha (2,200–3,600 lb/acre), and the U.S. and Brazil are the two largest soybean producers in the world. Due to the large production and trade of soybeans, approximately 11 million metric tons (24.6 billion lbs) of soybean oil were on the market in the 2016–2017 season; of that, 2.8 million metric tons (6.2 billion lbs) were used for biodiesel production (USDA ERS, 2018a).
In recent years, corn oil has been used increasingly and has become the second largest feedstock for making biodiesel in the U.S. Corn planted in the U.S. is mainly used for animal feed, corn starch or sweeteners, and for ethanol production. Corn oil can be extracted in a facility producing corn starch or sweeteners and is also increasingly being extracted from different byproducts of the ethanol industry. The total supply of corn oil in the U.S. was approximately 2.63 million metric tons (5.795 billion lbs) in 2017 (USDA ERS, 2018b). The quantity of corn oil used for biodiesel production was approximately 717,000 metric tons (1.579 billion lb), or approximately 10% of the total biodiesel market. Canola oil is the third largest feedstock with a use of approximately 659,000 metric tons (1.452 billion lbs) in 2017 (USDA EIA, 2018).
Rapeseed belongs to the Brassica family of oilseed crops. Original rapeseed, including the cultivars planted in China and India, contains very high contents of erucic acid and glucosinolates, chemicals undesirable in animal feed. Canola is a cultivar of rapeseed developed in Canada with very low erucic acid and glucosinolates contents. While the oilseed crop planted in Europe is still called rapeseed there, it is essentially the same plant called canola in North America. The yield of rapeseed in Europe is high, in the range of 2,000–3,500 kg/ha (1,800–3,100 lb/acre) and is planted almost exclusively for biodiesel production.
Other plant oils, including palm and coconut oil, can also be used for producing biodiesel and are especially popular in tropical nations due to very high oil yields per acre. Plant species with high oil yields, requiring low agricultural inputs and with the ability to grow on marginal lands, such as camelina and jatropha, are of particular interest and have been researched for biodiesel production. Oils from safflower, sunflower, and flaxseed can be used for making biodiesel, but their high value in the food industry makes them uneconomical for biodiesel production.
Some strains of microalgae have a high lipid content and are also widely researched and used to produce algal oil as a biodiesel feedstock. They are considered a promising feedstock because of their potential to be industrialized or produced in an industrial facility rather than on agricultural land. Microalgae can be cultivated in open ponds, but high-oil strains may be better suited to production in closed photo-bioreactors. The potential yield of microalgal oil per unit land can be as high as 6,000 L/ha/y (1600 gal/ac/y), more than 10 times that of canola or soybeans. Currently, however, microalgal lipids are not used for industrial biodiesel production because of their high production cost.
Like plant oils, animal fats contain similar chemical components and can be used directly for biodiesel production. In 2017, approximately 1.2 million metric tons (2.6 billion lbs) of used cooking oils and animal fats were used for biodiesel production in the U.S., accounting for 23% of the total used cooking oils and animal fats in the U.S. market (Swisher, 2018) and less than 20% of U.S. biodiesel production.
Conversion Process
Biodiesel is made by reacting triglycerides (the chemicals in oils and fats) with an alcohol. The chemical reaction is known as transesterification. In transesterification of oils and/or fats, which are the glycerol esters of fatty acids (Figure \(\PageIndex{2}\)), the glycerol needs to be transesterified by another alcohol, most commonly methanol. The three fatty acids (R1, R2, and R3) react with the alkyl groups of the alcohol to produce fatty acid esters, or biodiesel. Those fatty acids from the triglyceride are replaced by the hydroxyl groups from the alcohol to produce glycerol, a by-product. The glycerol can be separated from the biodiesel by gravity, but the process is typically accelerated through a centrifugation step. If methanol (CH3–OH) is used as the alcohol for the transesterification reaction, methyl groups attach to the liberated triglyceride fatty acids (Rx–CH3), as illustrated in Figure \(\PageIndex{2}\). The resulting mixture after glycerol separation is referred to as fatty acid methyl esters (or FAME as commonly called in Europe), and biodiesel after further refining. Without the glycerol skeleton, the mixture of FAME is much less viscous than the original vegetable oil or animal fat, and its fuel properties are suitable for powering diesel engines.
The transesterification of oils and fats involves a series of three consecutive reactions. Each fatty acid group is separated from the glycerol skeleton and transesterified individually. The intermediate products are diglycerides (when two fatty acid groups remain on the glycerol backbone) and monoglycerides (when one fatty acid group remains on the glycerol backbone). Transesterification reactions are also reversible. The diglyceride and monoglyceride intermediate products can react with a free fatty acid and reform triglycerides and diglycerides, respectively, under certain conditions. The degree of reverse reaction depends on the chemical kinetics of transesterification and the reaction conditions. In practical application, approximately twice the stoichiometric methanol requirement is added in order to drive the forward reactions and to ensure more complete conversion of oils and fats into biodiesel. The excess methanol can be recovered and purified for reuse in the system.
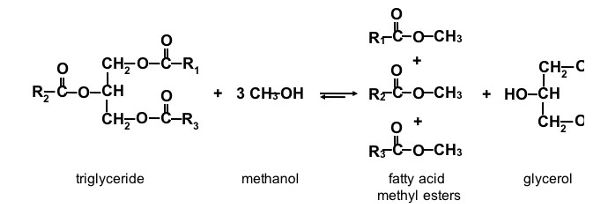
The density of vegetable oil at 25°C is in the range of 903–918 kg/m3 (7.53–7.65 lb/gal) depending on the specific feedstock (Forma et al., 1979). The density of biodiesel is approximately 870–880 kg/m3 (7.25–7.34 lb/gal) (Pratas et al., 2011). Comparison reveals that vegetable oil is approximately 4% heavier than biodiesel. While planning for biodiesel production, it is an acceptable assumption that each volume of biodiesel produced requires an equal volume of vegetable oil.
To calculate the exact volume of chemicals (i.e., reactant methanol and catalyst) needed for the transesterification, the molecular weight of the vegetable oil is needed. However, as seen from Table \(\PageIndex{3}\), vegetable oils vary in fatty acid composition depending on oil source and even on the specific plant cultivar. There is no defined molecular weight for all vegetable oil, but an average molecular weight is used for calculations. Based on the hydrolysis of fatty acid esters of glycerol, the molecular weight of vegetable oil (a mixture of fatty acid glycerol esters), MWave, can be calculated as:
\[ MW_{ave}=MW_{gly}\ -\ 3MW_{water} \ +\ 3MW_{ave,FA} \]
where MWgly = molecular weight of glycerol = 92.09 kg/kmol
MWwater = molecular weight of water = 18.02 kg/kmol
MWave,FA = average molecular weight of fatty acids in the oil
The water is subtracted in the equation because three individual fatty acids are joined to the single glycerol molecule in a condensation reaction that produces three water molecules in the process. The opposite reaction, hydrolysis, would split the fatty acid from the glycerol through incorporation of the water molecule ions into the products. The overall average molecular weight of vegetable oil fatty acids is calculated as:
\[ \frac{1}{MW_{ave,FA}}=\sum \frac{C_{i,FA}}{MW_{1,FA}} \]
where Ci,FA = mass fraction of a particular fatty acid
MWi,FA = molecular weight of that particular fatty acid
The difference between the weight of the methyl group (–CH3; 15 kg/kmol) and that of the hydrogen atom (–H; 1 kg/kmol) on the carboxyl group of fatty acids is 14 atomic mass units. To find the average molecular weight of fatty acid methyl esters (FAME) or biodiesel, MWave,FAME, the following formula can be used:
\[ MW_{ave,FAME}=MW_{ave,FA} \ + \ 14 \]

Use of a Catalyst
The transesterification reaction will occur even at room temperature if a vegetable oil is mixed with methanol, but would take an extraordinarily long time to approach equilibrium conditions. A catalyst and elevated temperatures are typically used to help the reaction move forward and dramatically reduce the reaction time. The catalysts suitable for transesterification of oils and fats are either strong acids or strong bases; the latter are most commonly used, especially for virgin vegetable oils. Sodium hydroxide (NaOH) and potassium hydroxide (KOH) are inexpensive choices for use as base catalysts; they are typically available commercially as solid flakes or pellets. Before being used as a catalyst for transesterification, the solid form of NaOH or KOH needs to be prepared by reacting with methanol to form a homogenous solution. This dissolving process is a chemical reaction to form soluble methoxide (–OCH3), as shown in Figure \(\PageIndex{3}\).
The methoxide is the active species for catalysis in the system. Therefore, the solution of sodium methoxide (NaOCH3) or potassium methoxide (KOCH3) in methanol are the preferred form of the catalysts for large continuous-flow biodiesel production. Solutions of NaOCH3 or KOCH3 in methanol are commercially available in 25–30% concentrations.
Other Factors Affecting Conversion
Note in Figure \(\PageIndex{3}\) that one mole of water is formed per mole of KOH reacted. Water in the transesterification of oils and/or fats is undesirable because it potentially leads to the hydrolysis of triglycerides to free fatty acids, which in turn react with the base catalyst, either KOH or KOCH3, to form soap. This soap-making process is called saponification (Figure \(\PageIndex{4}\)). Soap in the system will cause the reaction mixture to form a uniform emulsion, making the separation of biodiesel from its by-product glycerol impossible. Therefore, special attention is needed to avoid significant soap formation. Thus, prepared methoxide is preferred to hydroxide as the catalyst for use in biodiesel production, so water can be minimized in the system.
Transesterification of oils and/or fats requires a catalyst for realistic conversion rates, but the reaction will still take up to eight hours to complete if it is carried out at room temperature. Therefore, the process temperature also plays a very important role in the reaction rate, and higher reaction temperatures reduce the required reaction time. When the reaction temperature is maintained at 40°C (104°F), the time for complete transesterification can be shortened to 2–4 hours. If the reaction temperature is at 60°C (140°F), the time can be reduced even further to 1–2 hours for a batch reactor. The highest reaction temperature that can be applied under atmospheric pressure is limited by the boiling temperature of methanol, 64.5°C (148°F). Typical reaction temperatures for transesterification of oils and fats in large batch operations are in the range of 55–60°C (130–140°F). Higher temperatures can be used but require a closed system under pressure.

There are situations in which high amounts of free fatty acids (higher than 3% on a mass basis) exist naturally in feedstocks, such as used vegetable oils and microalgal lipids. To transesterify feedstocks with high free fatty acid content, direct application of base catalysts, either as hydroxide (–OH) or methoxide (–OCH3), is not recommended because of the increased likelihood of soap formation. Instead, a more complicated two-step transesterification process is used. In the first step, a strong acid, such as sulfuric acid (H2SO4), is used as a catalyst to convert most of the free fatty acids to biodiesel via a chemical process called esterification (Figure \(\PageIndex{5}\)). In the second step, a base catalyst is used to convert the remaining feedstock (mainly triglycerides) to biodiesel.

Safe Handling of Chemicals in Biodiesel Production
Conversion of oils and/or fats to biodiesel is a chemical reaction so a good understanding of the process chemistry, safe chemical processing practices, and all regulations is necessary to ensure safe and efficient biodiesel production. First aid stations must be in place in biodiesel laboratories and production facilities. Although biodiesel itself is a safe product to handle, some of the components involved in production can be hazardous. The chemicals in biodiesel production can include methanol, sodium or potassium hydroxide, and sulfuric acid, all of which have safety concerns related to storage and use. Extreme caution must be practiced in handling these chemicals during the whole process of biodiesel production. The appropriate Material Safety and Data Sheets for all chemicals used should be reviewed and followed to maintain personal and environmental safety.
Applications
Biodiesel Production Systems
The fundamental unit operations for transesterification of a feedstock with low free fatty acid content, such as virgin soybean or canola oil, using KOH as catalyst are illustrated in Figure \(\PageIndex{6}\). The catalyst solution is prepared by reacting it with methanol, in the case of hydroxide flakes, or by mixing it with a measured amount of methanol, in the case of methoxide solution, in a mixer. The prepared catalyst/methanol solution is added to the vegetable oil/fat in the reactor under gentle agitation. The reactor may be an individual or a series of stirred tanks, or some other reactor type. As discussed above, the transesterification reaction typically takes place in 1–2 hours at 55–60°C (130–140°F).
Crude glycerol is the term used for the glycerol fraction after initial separation. It contains some residual methanol, catalyst and a variety of other chemical impurities in the triglyceride feedstock. Crude glycerol is either refined on site or sold to a market for further processing. Although there are many uses of glycerol in industries from food to cosmetics to pharmaceuticals, the economics of refining severely limits its use. The grey water from biodiesel washing is a waste product containing small quantities of methanol, glycerol, and catalyst. It needs adequate treatment before it can be discharged to a municipal wastewater system.
Process Configuration
Biodiesel can be produced in a batch, semi-continuous, or continuous process. The economics of process configuration are largely dependent on production capacity. Batch processes require less capital investment and are easier to build. A major advantage of batch processing is the flexibility to accommodate variations in types and quantities of feedstock. Challenges of batch processing include lower productivity, higher labor needs, and inconsistent fuel quality. Continuous-flow biodiesel production processes can be scaled more easily and are preferred by larger producers. In continuous-flow processes, fuel quality is typically very consistent. The higher initial capital costs, including costs for complicated process control and process monitoring, are mitigated in large operations by greater throughput and higher quality product. As a result, the net capital and operating costs per unit product is less than that of batch processes. The types of reactors for transesterification can be simple stirred tanks for batch processes and continuously stirred tank reactors (CSTR) for continuous-flow processes.
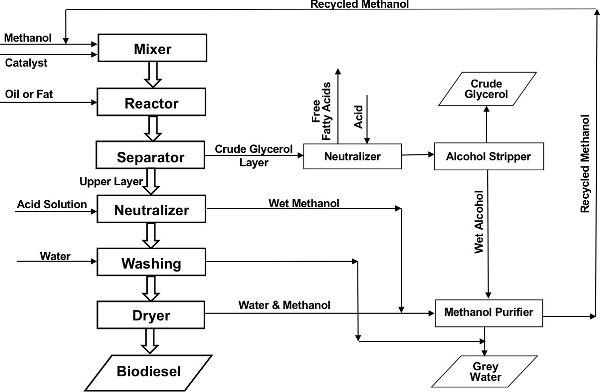
Upon completion of the reaction, the product mixture passes to a separator, which can be a decanter for a batch process or a centrifuge for the continuous-low system. The crude glycerol, which is denser than the biodiesel layer, is removed. Any residual catalyst in the biodiesel layer is then neutralized by a controlled addition of an acid solution. In the same unit, most of the excess methanol and some residual glycerol is concentrated in the aqueous acid solution layer and withdrawn to a methanol recovery unit, where the methanol is concentrated, purified, and recirculated for reuse.
The neutralized biodiesel layer is washed by gentle contact with softened water to further remove residual methanol and glycerol. The washed biodiesel layer is dried by heating to approximately 105°C (220°F) until all moisture is volatized. The finished biodiesel after drying is tested for quality before being transferred to storage tanks for end use or distribution.
Biodiesel Storage and Utilization
Biodiesel has relatively low thermal and oxidative stabilities. This is due to the unsaturated double bonds contained in the oil and fat feedstocks. Therefore, biodiesel should be stored in cool, light-proof containers, preferably in underground storage facilities. The storage containers should be semi-sealed to minimize air exchange with the environment, reducing the possibility of oxidation and moisture absorption of biodiesel. Where permitted, the headspace of the storage containers can be filled with nitrogen to prevent biodiesel from coming into contact with oxygen. If biodiesel will be stored for longer than six months before use, adding a biocide and a stability additive is necessary to avoid microbial activity in the biodiesel. Biodiesel storage and transportation containers should not be made of aluminum, bronze, copper, lead, tin, or zinc because contact with these types of metals will accelerate degradation. Containers made of steel, fiberglass, fluorinated polyethylene, or Teflon can be used.
Biodiesel is a much stronger solvent than conventional diesel. Storage tanks for conventional diesel may have organic sludge build-up in them. If using such tanks for biodiesel storage, they should be thoroughly cleaned and dried to prevent the sludge from being dissolved by biodiesel and potentially causing problems to fuel lines and fuel filters. Similar problems can occur when using biodiesel in older engines with petroleum residues in fuel tanks or transfer lines. For more information on handling and storing biodiesel, readers are recommended to consult “Biodiesel Handling and Use Guide” (5th ed.) prepared by the National Renewable Energy Laboratory of the U.S. Department of Energy (Alleman et al., 2016).
Examples
Example \(\PageIndex{1}\)
Example 1: Volumes of soybean oil for biodiesel production
Problem:
Last year, a farmer used a total of 13,250 L of diesel fuel to run the farm’s machinery and trucks. After attending a workshop on using biodiesel on farms for both economic and environmental benefits, the farmer has decided to use a B20 blend of biodiesel in all the farm’s vehicles. The average annual yield of soybeans on the farm is 2,800 kg/ha. The soybeans contain 18.5% oil on a mass basis, and the efficiency of soybean oil extraction through mechanical pressing is approximately 80%. The density of soybean oil is 916 kg/m3.
Answer the following questions to help the farmer develop the details needed:
- (a) How much pure biodiesel (B100) is needed to run the farm’s vehicles using a B20 blend (i.e., a mixture of 20% biodiesel and 80% of conventional diesel on a volume basis)?
- (b) How much soybean oil is needed to produce sufficient B100 to blend with conventional diesel?
- (c) What field area will yield enough soybeans for the needed quantity of oil?
Solution
- (a) Given that the farmer uses 13,250 L of diesel fuel yearly, if 20% of the quantity is replaced by biodiesel, the quantity of pure biodiesel must be:
\( 13,250 \ \text{L} \times0.20=2,650 \ \text{L} \)
- The farmer will still need to purchase conventional diesel fuel, which is 80% of the total consumption:
\( 13,250 \ \text{L} \times0.80=2,650 \ \text{L} \)
- Therefore, 2,650 L of pure biodiesel (B100) is needed to blend with 10,600 L of conventional diesel to make a total of 13,250 L of a B20 blend for the farm’s vehicles.
- (b) As an estimate of how much soybean oil (in kg) is needed, each volume of biodiesel requires approximately one volume of soybean oil (or other oil) to produce it, as noted in the Conversion Process section. Therefore, the initial estimate for the quantity of soybean oil is the same as the required quantity of pure biodiesel, i.e., 2,650 L of soybean oil.
Calculate the mass quantity of soybean oil by multiplying the volume of soybean oil by the density of soybean oil (916 kg/m3 or 0.916 kg/L):
\( 2,650 \ \text{L} \times0.916 \frac{\text{kg}}{\text{L}}=2,427 \ \text{kg} \)
- (c) The given soybean yield is 2,800 kg/ha, the oil content of soybean is 18.5%, and the oil extraction efficiency is 80%. Therefore, each ha planted in soybean will yield:
(2800 kg) (0.185) (0.80) = 414.4 kg of soybean oil
- The area of soybean field for produce the needed 2427 kg of soybean oil is:
2,427 kg / 414.4 kg/ha = 5.86 ha
In summary, the farmer needs to plant at least 5.86 ha of soybeans to have enough soybean oil to produce the biodiesel needed to run the farm’s vehicles.
Example \(\PageIndex{2}\)
Example 2: Average molecular weight of soybean oil
Problem:
The farmer had the farm’s soybean oil analyzed by a commercial laboratory via a gas chromatographic analysis and obtained the following fatty acid profile on a mass basis:
Palmitic (C16:0) | Stearic (C18:0) | Oleic (C18:1) | Linoleic (C18:2) | Linolenic (C18:3) | |
---|---|---|---|---|---|
Profile |
9% |
4% |
22% |
59% |
6% |
MWi,FA (kg/kmol) |
256.5 |
284.5 |
282.5 |
280.5 |
278.5 |
- (a) What is the average molecular weight of the soybean oil?
- (b) What is the average molecular weight of biodiesel from this soybean oil?
Solution
- (a) First, calculate the average molecular weight of fatty acids (MWave,FA) in the soybean oil using Equation 1.3.2:
- \( \frac{1}{MW_{ave,FA}} = \sum\frac{C_{i,FA}}{MW_{i,FA}} \) (Equation \(\PageIndex{2}\))
- \( \frac{1}{MW_{ave,FA}} = \frac{9 \%}{256.5}+\frac{4 \%}{284.5}+\frac{22 \%}{282.5}+\frac{59 \%}{280.5}+\frac{6 \%}{278.5} \)
- \( = \frac{0.09 \%}{256.5}+\frac{0.04 \%}{284.5}+\frac{0.22 \%}{282.5}+\frac{0.59 \%}{280.5}+\frac{0.06 \%}{278.5} \)
= 0.003589 kmol/kg
- Therefore, MWave,FA = 1 / 0.003589 = 278.6 kg/kmol.
- Next, calculate the average molecular weight of the soybean oil using Equation 1.3.1:
- \( MW_{ave}=MW_{gly}-3MW_{water}+3MW_{ave,FA} \) (Equation \(\PageIndex{1}\))
\( = 92.09 - (3 \times18.02)+(3 \times278.6) \frac{kg}{kmol} \)
Therefore, MWave= 873.7 kg/kmol.
- (2) Calculate the average molecular weight of biodiesel using Equation 1.3.3:
- \( MW_{ave,FAME}=MW_{ave,FA}+14 \) (Equation \(\PageIndex{3}\))
\( =278.6+14\frac{kg}{kmol} \) (Equation \(\PageIndex{3}\))
Therefore, MWave,FAME = 292.6 kg/kmol.
In summary, the average molecular weights of the soybean oil and biodiesel are 873.7 and 292.6 kg/kmol, respectively.
Example \(\PageIndex{3}\)
Example 3: Chemicals in converting soybean oil to biodiesel
Problem:
As determined in Example 1.3.1, the farmer needs to produce 2,650 L of pure biodiesel (B100; molecular weight = 880 kg/kmol) to run the farm’s vehicles and machinery with B20 blends. In converting soybean oil into biodiesel, the methanol (CH3OH, molecular weight = 32.04 kg/kmol) application rate needs to be 100% more than the stoichiometrically required rate to ensure a complete reaction. The application rate of the potassium hydroxide catalyst (KOH, molecular weight = 56.11 kg/kmol) is 1% of the soybean oil on a mass basis. How much methanol and potassium hydroxide, in kg, are needed to produce the required biodiesel? The average molecular weights of the soybean oil and biodiesel are 873.7 and 292.6 kg/kmol, respectively.
Solution
First, write out the transesterification of soybean oil to biodiesel with known molecular weights (MW) (similar to Figure 1.3.2):
Next, convert the quantity of biodiesel from volume to mass by the biodiesel density, 880 kg/m3 = 0.880 kg/L:
\( 2,650 \text{ L} \times 0.880 \frac{\text kg}{\text L} = 2,332 \text{ kg} \)
Next, calculate the amount of methanol from the stoichiometric ratio of the transesterification reaction.
methanol : biodiesel
- The stoichiometric mass ratio 3 × 32.04 : 3 × 292.6
- The unknown mass ratio (kg) M : 2,332
- Or (3 × 292.6) × M = (3 × 32.04) × 2,332
Therefore, the quantity of methanol is
\( M = (3 \times 32.04)\times2,332\text{kg} / (3 \times 292.6) =255.5 \text{kg} \)
Next, calculate the total amount of methanol with 100% excess, as required:
\( M’ = 2M = 2 \times 255.5 = 511 \text{ kg} \)
Finally, calculate the quantity of catalyst KOH needed. Since the application rate of the catalyst KOH is 1% of the soybean oil, before the quantity of KOH can be calculated, the quantity of soybean oil must be obtained from the stoichiometric ratio of the transesterification reaction.
soybean oil : biodiesel
- The stoichiometric mass ratio 873.7 : 3 × 292.6
- The unknown mass ratio (kg) S : 2,332
- Or (3 × 292.6) × S = 873.7 × 2,332 kg
The quantity of soybean oil is, then:
\( S = 873.7 \times 2,332 \text{ kg} / (3 \times 292.6) = 2,321 \text{ kg} \)
Therefore, the quantity of catalyst KOH is calculated as 1% of the oil:
\( 2,332 \text{ kg} \times 0.01 = 23.2 \text{ kg} \)
In summary, the quantities of methanol and potassium hydroxide are 511 kg and 23.2 kg, respectively.
Image Credits
Figure 1. He, B. (CC By 4.0). (2020). Chemical structure of triglycerides, glycerol, and fatty acids. R, R1, R2, and R3 represent alkyl groups typically with carbon chain lengths of 15–17 atoms.
Figure 2. He, B. (CC By 4.0). (2020). Transesterification of triglycerides with methanol. R1, R2, and R3 are alkyl groups in chain lengths of, most commonly, 15–17 carbons.
Figure 3. He, B. (CC By 4.0). (2020). Chemical reaction between methanol and potassium hydroxide to form potassium methoxide.
Figure 4. He, B. (CC By 4.0). (2020). Saponification between potassium hydroxide and a fatty acid.
Figure 5. He, B. (CC By 4.0). (2020). Esterification of a fatty acid reacting with methanol (in the presence of an acid catalyst) to yield a methyl ester and water.
Figure 6. He, B. (CC By 4.0). (2020). Schematic illustration of a biodiesel production system.
The chemical formula in Example 3. He, B. (CC By 4.0). (2020).
References
Alleman, T. L., McCormick, R. L., Christensen, E. D., Fioroni, G., Moriarty, K., & Yanowitz, J. (2016). Biodiesel handling and use guide (5th ed.). Washington, DC: National Renewable Energy Laboratory, U.S. Department of Energy. DOE/GO-102016-4875. https://doi.org/10.2172/1332064.
ASTM. (2015). D6751-15ce1: Standard specification for biodiesel fuel blend stock (B100) for middle distillate fuels. West Conshohocken, PA: ASTM Int. https://doi.org/10.1520/D6751-15CE01.
ASTM, D975. (2019). D975-19c: Standard specification for diesel fuel. West Conshohocken, PA: ASTM Int. https://doi.org/10.1520/D0975-19C.
Canakci, M., & Sanli, H. (2008). Biodiesel production from various feedstocks and their effects on the fuel properties. J. Ind. Microbiol. Biotechnol., 35(5), 431-441. https://doi.org/10.1007/s10295-008-0337-6.
CEN. (2013). EN14214+A1 Liquid petroleum products—Fatty acid methyl esters (FAME) for use in diesel engines and heating applications—Requirements and test methods. Brussels, Belgium: European Committee for Standardization.
Dubois, V., Breton, S., Linder, M., Fanni, J., & Parmentier, M. (2007). Fatty acid profiles of 80 vegetable oils with regard to their nutritional potential. European J. Lipid Sci. Technol., 109(7), 710-732. doi.org/10.1002/ejlt.200700040.
Forma, M. W., Jungemann, E., Norris, F. A., & Sonntag, N. O. (1979). Bailey’s industrial oil and fat products. D. Swern (Ed.), (4th ed., Vol. 1, pp 186-189). New York, NY: John Wiley & Sons.
Goodrum, J. W., & Geller, D. P. (2005). Influence of fatty acid methyl esters from hydroxylated vegetable oils on diesel fuel lubricity. Bioresour. Technol., 96(7), 851-855. https://doi.org/10.1016/j.biortech.2004.07.006.
He, B. B., Thompson, J. C., Routt, D. W., & Van Gerpen, J. H. (2007). Moisture absorption in biodiesel and its petro-diesel blends. Appl. Eng. Agric., 23(1), 71-76. https://doi.org/10.13031/2013.22320.
Knothe, G., Krabl, J., & Van Gerpen, J. (2015). The biodiesel handbook (2nd ed.). AOCS Publ.
Kostik, V., Memeti, S., & Bauer, B. (2013). Fatty acid composition of edible oils and fats. J. Hygienic Eng. Design, 4, 112-116. Retrieved from http://eprints.ugd.edu.mk/11460/1/06.%20Full%20paper%20-%20Vesna%20Kostik%202.pdf.
Peterson, C. L. (1986). Vegetable oil as a diesel fuel: Status and research priorities. Trans. ASAE, 29(5), 1413-1422. https://doi.org/10.13031/2013.30330.
Peterson, C. L., Wagner, G. L., & Auld, D. L. (1983). Vegetable oil substitutes for diesel fuel. Trans. ASAE, 26(2), 322-327. https://doi.org/10.13031/2013.33929.
Prasad, S., & Ingle, A. P. (2019). Chapter 12. Impacts of sustainable biofuels production from biomass. In M. Rai, & A. P. Ingle (Eds.), Sustainable bioenergy—Advances and impacts (pp. 327-346). Cambridge, MA: Elsevier. https://doi.org/10.1016/B978-0-12-817654-2.00012-5.
Pratas, M. J., Freitas, S. V., Oliveira, M. B., Monteiro, S. l. C., Lima, A. l., & Coutinho, J. A. (2011). Biodiesel density: Experimental measurements and prediction models. Energy Fuels, 25(5), 2333-2340. https://doi.org/10.1021/ef2002124.
Sheehan, J., Camobreco, V., Duffield, J., Graboski, M., & Shapouri, H. (1998). Life cycle inventory of biodiesel and petroleum diesel for use in an urban bus. NREL/SR-580-24089. Golden, CO: National Renewable Energy Laboratory. https://doi.org/10.2172/658310.
Swisher, K. (2018). U.S. market report: Fat usage up but protein demand down. Render Magazine. Retrieved from http://www.rendermagazine.com/articles/.
USDA EIA. (2018). Monthly biodiesel production report. Table 3, U.S. inputs to biodiesel production. Washington, DC: USDA EIA. Retrieved from www.eia.gov/biofuels/biodiesel/production/.
USDA ERS. (2018a). U.S. bioenergy statistics. Table 6, Soybean oil supply, disappearance and share of biodiesel use. Washington, DC: USDA ERS. Retrieved from https://www.ers.usda.gov/data-products/us-bioenergy-statistics/.
USDA ERS. (2018b). U.S. bioenergy statistics. Table 7, Oils and fats supply and prices, marketing year. Washington, DC: USDA ERS. Retrieved from https://www.ers.usda.gov/data-products/us-bioenergy-statistics/.